# Creating Stronger Magnets: Exploring Novel Materials for Enhanced Magnetic Fields
**Summary:** This article explores the ongoing quest for stronger magnets, delving into the limitations of current materials like neodymium and samarium cobalt, and examining promising next-generation materials that could revolutionize industries relying on high-strength magnets. We will investigate potential candidates, the challenges in their development, and the impact these advancements could have on various applications, from electric vehicles to medical imaging. Read on to discover the future of magnetism and the materials poised to power it.
## The Limitations of Current Strong Magnet Materials
Neodymium magnets (NdFeB) and Samarium Cobalt magnets (SmCo) currently reign supreme in high-performance applications. However, they aren’t without limitations. Neodymium magnets, while possessing superior strength compared to SmCo, suffer from a significant drawback: their Curie temperature. Above this temperature, the magnet loses its magnetism, rendering it useless. SmCo magnets, while having a higher Curie temperature, are more brittle and expensive to manufacture. Furthermore, the reliance on rare earth elements in both materials presents supply chain vulnerabilities and environmental concerns related to mining and processing. The demand for even stronger and more stable magnets is growing, driving research into alternatives.
The coercive force, a measure of a magnet’s resistance to demagnetization, is another critical parameter. Both NdFeB and SmCo magnets can be susceptible to demagnetization under adverse conditions, such as high temperatures or strong opposing magnetic fields. This limits their use in certain demanding applications. Moreover, the performance of NdFeB magnets degrades over time due to corrosion, necessitating protective coatings that add to the cost and complexity of manufacturing. The industry needs magnets that retain their strength and stability over a longer lifespan and across a wider range of operating conditions.
Finally, the theoretical performance limits of current magnet materials are being approached. Simply refining existing techniques will only yield marginal improvements. A significant leap forward requires the discovery and development of entirely new magnetic materials with fundamentally different properties. This entails exploring alternative compositions, crystal structures, and manufacturing processes to overcome the inherent limitations of NdFeB and SmCo magnets.
## The Quantum Leap: Beyond Traditional Magnet Design
Moving beyond simply optimizing existing materials requires embracing novel approaches to magnet design, often drawing inspiration from quantum mechanics and advanced materials science. This involves exploring novel crystal structures, manipulating electron spin, and utilizing complex chemical compositions to engineer magnetic properties at the atomic level. The goal is to create materials with significantly higher magnetic anisotropy, exchange coupling, and Curie temperatures than presently achievable.
One promising avenue is the investigation of materials exhibiting topological magnetism. These materials possess unique electronic structures that give rise to unusual magnetic phenomena, such as skyrmions and magnetic Weyl fermions. These topologically protected magnetic textures offer the potential for ultra-stable and energy-efficient magnetic storage and spintronic devices, which could indirectly enhance the performance of magnets used in various applications. Exploring and harnessing these quantum phenomena represents a fundamental shift in the approach to magnet development.
Furthermore, computational materials science plays an increasingly crucial role in the search for new magnet materials. Sophisticated simulations can predict the magnetic properties of hypothetical materials before they are even synthesized, drastically accelerating the discovery process. By screening vast chemical spaces and identifying promising candidates based on theoretical calculations, researchers can focus their experimental efforts on the most likely prospects, saving time and resources. This approach enables the rapid exploration of a much wider range of potential materials.
## Manganese-Based Compounds: A Promising Alternative
Manganese-based compounds are emerging as a potential alternative to rare-earth magnets due to their abundance and relatively low cost. These materials, often containing elements such as aluminum, carbon, and iron in addition to manganese, exhibit intriguing magnetic properties that are being actively investigated. The challenge lies in optimizing their composition and crystal structure to achieve the desired combination of high saturation magnetization, coercive force, and Curie temperature.
One specific example is MnBi (Manganese Bismuth). This compound exhibits significant uniaxial magnetocrystalline anisotropy, a crucial factor for achieving high coercive force and preventing demagnetization. However, MnBi presents challenges related to its synthesis and phase stability. Researchers are exploring novel processing techniques to improve its magnetic properties and make it more suitable for practical applications. Other manganese-based alloys, such as MnAl and MnGa, are also under investigation, each with its own unique set of advantages and drawbacks.
The appeal of manganese-based compounds extends beyond their cost and availability. They offer the potential for creating environmentally friendly magnets that do not rely on rare earth elements. This is particularly important in light of growing concerns about the environmental impact of mining and processing rare earth ores. Research into manganese-based magnets is a crucial step towards a more sustainable future for the magnetic materials industry.
## Iron-Based Superlattices: Engineering Magnetism Layer by Layer
Iron-based superlattices represent a different approach to creating stronger magnets, focusing on the precise control of atomic layering to engineer specific magnetic properties. These structures consist of alternating layers of different materials, carefully chosen to enhance the desired magnetic characteristics. By controlling the thickness and composition of each layer, researchers can tailor the magnetic anisotropy, exchange coupling, and Curie temperature of the resulting material.
One prominent example is the creation of Fe/X multilayers, where Fe represents iron and X is a non-magnetic element such as platinum (Pt) or palladium (Pd). The interactions between the iron layers and the non-magnetic layers can induce perpendicular magnetic anisotropy (PMA), where the magnetization is preferentially oriented perpendicular to the plane of the layers. PMA is crucial for achieving high coercive force and enabling the development of high-density magnetic recording media.
The challenge lies in achieving precise control over the layer thickness and interface quality during the fabrication process. Techniques such as molecular beam epitaxy (MBE) and sputtering are commonly used to create superlattices with atomic-level precision. However, these techniques can be complex and expensive. Researchers are exploring new deposition methods and materials combinations to further improve the performance and scalability of iron-based superlattices.
## High-Entropy Alloys: Unleashing Complexity for Enhanced Performance
High-entropy alloys (HEAs) are a relatively new class of materials that contain multiple elements in approximately equal proportions. This compositional complexity can lead to unique microstructures and properties, including enhanced magnetic performance. The presence of multiple elements disrupts the conventional crystal lattice, creating a highly distorted environment that can enhance magnetic anisotropy and exchange coupling.
The beauty of HEAs lies in their vast compositional space. By carefully selecting and combining different elements, researchers can fine-tune the magnetic properties of the alloy to meet specific application requirements. For example, HEAs containing iron, cobalt, nickel, and other transition metals have shown promising results in terms of saturation magnetization, coercive force, and Curie temperature.
However, the complexity of HEAs also presents challenges. The large number of possible compositions makes it difficult to predict the properties of a given alloy. Computational modeling and machine learning are being used to accelerate the discovery process by identifying promising HEA compositions and predicting their magnetic behavior. Optimizing the processing parameters, such as annealing temperature and cooling rate, is also crucial for achieving the desired microstructure and magnetic properties.
## Nanocomposite Magnets: Combining Hard and Soft Magnetic Phases
Nanocomposite magnets leverage the synergistic effects of combining hard and soft magnetic phases at the nanoscale. The hard magnetic phase provides high coercive force, while the soft magnetic phase contributes to high saturation magnetization. By carefully controlling the size, shape, and distribution of the two phases, researchers can create magnets with superior energy product, a measure of the overall magnetic performance.
A typical nanocomposite magnet consists of hard magnetic grains embedded in a soft magnetic matrix. The exchange coupling between the two phases allows the soft magnetic phase to enhance the remanence of the hard magnetic phase, leading to a significant increase in the energy product. The size of the hard magnetic grains must be smaller than the domain wall width of the soft magnetic phase to ensure effective exchange coupling.
Creating nanocomposite magnets with optimized microstructure requires careful control over the processing parameters. Techniques such as melt spinning, mechanical alloying, and severe plastic deformation are commonly used to create the desired microstructure. Researchers are also exploring new materials combinations and processing techniques to further improve the performance of nanocomposite magnets. For instance, combining rare-earth-free hard magnetic phases with iron-based soft magnetic phases can lead to cost-effective and environmentally friendly high-performance magnets.
## Additive Manufacturing: Tailoring Magnet Shapes and Properties
Additive manufacturing, also known as 3D printing, offers a powerful tool for creating magnets with complex shapes and tailored magnetic properties. By selectively depositing layers of material, additive manufacturing allows for the fabrication of magnets with geometries that are impossible to achieve using conventional manufacturing techniques. This opens up new possibilities for designing magnets with optimized performance and integrating them directly into devices.
One of the key advantages of additive manufacturing is the ability to control the microstructure of the magnet during the printing process. By adjusting the printing parameters, such as laser power, scan speed, and layer thickness, researchers can influence the grain size, texture, and phase distribution of the magnet. This allows for the creation of magnets with customized magnetic anisotropy and exchange coupling.
However, additive manufacturing of magnets also presents challenges. The high temperatures involved in the printing process can lead to oxidation and degradation of the magnetic properties. Furthermore, the surface finish of additively manufactured magnets can be rough, requiring post-processing steps to improve their magnetic performance. Researchers are actively developing new materials and printing techniques to overcome these challenges and unlock the full potential of additive manufacturing for magnet fabrication.
## The Future of Magnetic Materials: Applications and Implications
The development of stronger and more sustainable magnets will have a profound impact on a wide range of applications, including electric vehicles (EVs), renewable energy, medical imaging, and data storage. In the EV industry, stronger magnets are needed to improve the performance and efficiency of electric motors. Smaller and lighter motors can extend the driving range of EVs and reduce their overall weight. Similarly, more powerful magnets are essential for wind turbines and other renewable energy systems, enabling them to generate more electricity with higher efficiency.
In the medical field, stronger magnets are crucial for improving the resolution and sensitivity of MRI scanners. This allows for earlier and more accurate diagnosis of diseases. Stronger magnets are also being used in targeted drug delivery systems, enabling precise and localized treatment of various conditions. Furthermore, advancements in magnetic data storage technology are driven by the need for higher storage densities and faster data access speeds. Stronger and more stable magnets are essential for achieving these goals.
The quest for stronger magnets is not just a scientific endeavor; it is a critical component of technological progress and sustainable development. The development of new magnetic materials will not only enhance the performance of existing technologies but also enable the creation of entirely new applications that are currently unimaginable. Investing in research and development in this field is essential for securing a brighter and more sustainable future.
## Conclusion
The pursuit of stronger magnets is a continuously evolving field, driven by the limitations of current materials and the ever-increasing demands of advanced technologies. While neodymium and samarium cobalt magnets have dominated the landscape for years, their reliance on rare earth elements, limited thermal stability, and approaching theoretical performance limits necessitate the exploration of novel materials. Manganese-based compounds offer a promising alternative due to their abundance and lower cost, while iron-based superlattices provide fine-tuned control over magnetic properties through engineered layered structures. High-entropy alloys leverage compositional complexity to enhance magnetic performance, and nanocomposite magnets combine hard and soft magnetic phases for superior energy product. Additive manufacturing adds another dimension by enabling the creation of complex shapes and tailored properties. These advancements, fueled by computational modeling and innovative fabrication techniques, promise to revolutionize industries ranging from electric vehicles and renewable energy to medical imaging and data storage. The future of magnetic materials lies in a multifaceted approach, embracing both incremental improvements to existing materials and the radical exploration of new compositions and architectures.
## FAQ
### What are the main limitations of current strong magnet materials like neodymium magnets?
Neodymium magnets, while strong, have a relatively low Curie temperature, meaning they lose their magnetism at moderately high temperatures. They’re also vulnerable to corrosion and rely on rare earth elements, leading to supply chain and environmental concerns.
### What materials are being explored as alternatives to rare earth magnets?
Researchers are actively investigating manganese-based compounds, iron-based superlattices, and high-entropy alloys as potential alternatives to rare earth magnets. These materials offer the potential for cost-effectiveness, sustainability, and enhanced performance.
### What is the role of computational materials science in the search for new magnet materials?
Computational materials science plays a crucial role by predicting the magnetic properties of hypothetical materials before they are synthesized, significantly accelerating the discovery process and saving valuable resources.
### How can additive manufacturing be used to improve magnets?
Additive manufacturing, or 3D printing, allows for the creation of magnets with complex shapes and tailored magnetic properties by controlling the microstructure during the printing process. This enables optimized performance and direct integration into devices.
### What are nanocomposite magnets and how do they work?
Nanocomposite magnets combine “hard” and “soft” magnetic phases at the nanoscale. The hard phase provides high coercive force (resistance to demagnetization), while the soft phase contributes high saturation magnetization (magnetic strength). When combined correctly, the properties of both magnify in the resulting material.
### What are some of the key applications that will benefit from stronger magnets?
Electric vehicles, renewable energy systems, medical imaging (MRI), and data storage are just some of the key applications that will benefit from stronger and more efficient magnets. They’ll allow for smaller, lighter, and more powerful devices in all those spaces.
### What is magnetic anisotropy?
Magnetic anisotropy refers to the directional dependence of a material’s magnetic properties. In the context of magnets, anisotropy implies that the material is easier to magnetize along a particular direction (easy axis) than along others. High magnetic anisotropy is important because it helps prevent demagnetization.
### What is “Curie temperature” and why is it important?
The Curie temperature is the temperature at which a ferromagnetic material loses its ferromagnetism and becomes paramagnetic. Below the Curie temperature, the material can sustain a magnetic field. High Curie temperature is desirable because it means the magnet can operate at higher temperatures without losing its magnetisation.
### How does the environmental impact of new magnet materials compare to that of existing ones?
The focus on manganese-based compounds and other rare-earth-free options is, in part, driven by a desire to create more sustainable magnets. The environmental impact of mining and processing rare earths is significant, and finding viable alternatives would greatly reduce the industry’s overall footprint. New materials could also be more durable, reducing replacements and waste.
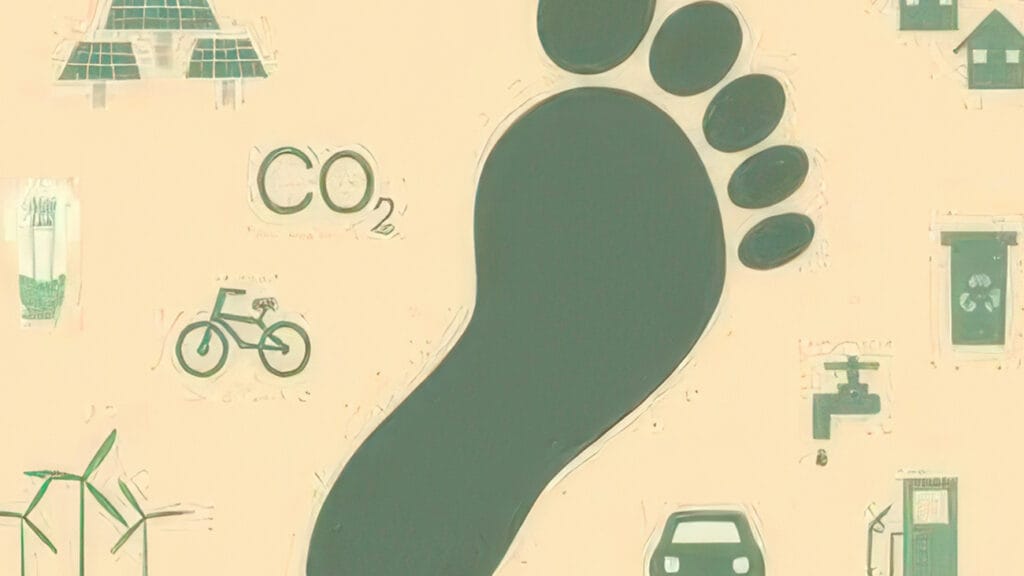