# Revolutionizing Technology: A Deep Dive into Recent Magnetic Material Breakthroughs
**Summary:** This article explores the cutting edge of magnetic material science, highlighting recent breakthroughs with game-changing potential. From novel permanent magnets surpassing current performance limits to advanced magnetocaloric materials for efficient cooling, and metamaterials enabling unprecedented control over magnetic fields to spintronic devices paving the way for faster and more energy-efficient computing, we delve into the innovations that are shaping the future of technology. This comprehensive overview is essential for anyone interested in materials science, physics, engineering, or the future of technology.
## I. Novel Permanent Magnets: Pushing the Boundaries of Performance
The quest for stronger, more efficient, and less resource-intensive permanent magnets is a constant driving force in magnetic materials research. Rare-earth magnets, particularly neodymium-iron-boron (NdFeB) magnets, have dominated the market for decades due to their exceptional energy product (a measure of magnetic strength). However, reliance on scarce and geographically concentrated rare-earth elements has spurred the search for alternatives and improvements. Recent advancements focus on two primary avenues: enhancing the performance of rare-earth magnets and developing rare-earth-free magnets with comparable or even superior properties.
One promising approach involves tweaking the microstructure of NdFeB magnets. Researchers are exploring techniques like grain boundary diffusion and alloying to optimize the alignment of magnetic domains and prevent their demagnetization at high temperatures. These efforts have led to incremental improvements in the maximum energy product and coercivity (resistance to demagnetization) of NdFeB magnets, extending their applicability to more demanding applications such as electric vehicle motors and wind turbine generators. Simultaneously, scientists are investigating novel architectures, such as exchange-spring magnets, which combine hard and soft magnetic phases to enhance the overall magnetic performance.
The second significant thrust is the development of rare-earth-free permanent magnets. Intermetallic compounds containing abundantly available elements like iron, cobalt, nickel, and manganese are being actively investigated. For instance, certain iron-nitride (FeN) compounds have shown considerable potential, although their practical implementation is still challenging due to their metastability and complex synthesis requirements. Another promising class of materials involves high-anisotropy iron-based alloys containing additives like carbon or boron. These materials, while not yet matching the performance of NdFeB magnets, offer a viable pathway to reducing dependence on rare earths and improving the sustainability of magnetic technology.
## II. Magnetocaloric Materials: Innovations for Efficient and Green Cooling
Magnetocaloric materials exhibit a change in temperature when exposed to a changing magnetic field, a phenomenon known as the magnetocaloric effect (MCE). This effect can be harnessed for magnetic refrigeration, offering a more energy-efficient and environmentally friendly alternative to traditional vapor-compression cooling systems. Current research in magnetocaloric materials focuses on identifying and developing materials with large MCEs, operating near room temperature, and exhibiting good thermal conductivity and mechanical stability.
Gadolinium (Gd) was one of the first materials recognized for its substantial MCE near room temperature, and it remains a benchmark for comparison. However, Gd is expensive and exhibits a relatively narrow operating temperature range. As such, researchers are exploring a wide range of alternative materials, including alloys, intermetallic compounds, and metal-organic frameworks (MOFs). Alloys based on lanthanum, iron, manganese, and silicon (LaFeMnSi) have shown significant promise due to their large MCEs and relatively low cost. However, their performance can be sensitive to processing conditions and compositional variations.
Ongoing research efforts focus on optimizing the composition and microstructure of these alloys to enhance their MCEs and broaden their operating temperature range. Researchers are also investigating new materials, such as Heusler alloys (e.g., NiMnGa), which exhibit martensitic phase transitions that can be manipulated by magnetic fields to produce significant MCEs. The development of high-performing magnetocaloric materials is crucial for realizing the full potential of magnetic refrigeration technology, paving the way for more efficient and sustainable cooling solutions in various applications, from domestic refrigerators to industrial cooling systems.
## III. Soft Magnetic Materials: Enabling High-Frequency Applications
Soft magnetic materials are characterized by their high permeability and low coercivity, making them ideal for applications requiring rapid magnetization and demagnetization, such as transformers, inductors, and magnetic shielding. The performance of soft magnetic materials is critical for the efficiency and miniaturization of these devices, particularly in high-frequency applications. Research in soft magnetic materials focuses on reducing core losses (energy dissipated as heat during magnetization and demagnetization cycles) and enhancing the saturation magnetization (the maximum magnetic moment that a material can achieve).
Traditional soft magnetic materials, such as silicon steel and ferrites, have been widely used for decades. However, their performance can be limited in high-frequency applications due to eddy current losses and relatively low saturation magnetization. Researchers are exploring novel soft magnetic materials with improved properties, including nanocrystalline alloys, amorphous alloys, and composite materials. Nanocrystalline alloys, such as Finemet (Fe-Si-B-Nb-Cu), exhibit exceptionally high permeability and low core losses due to their fine grain size, which reduces eddy current pathways and enhances domain wall motion.
Amorphous alloys, lacking long-range crystalline order, also offer excellent soft magnetic properties, including high permeability and low coercivity. These materials can be produced by rapid solidification techniques, such as melt spinning, and are particularly well-suited for high-frequency applications. Composite materials, consisting of soft magnetic particles embedded in a non-magnetic matrix, offer a versatile platform for tailoring magnetic properties and minimizing core losses. These materials can be designed to exhibit high resistivity, which suppresses eddy current losses, and can be easily shaped into complex geometries.
## IV. Magnetic Metamaterials: Controlling Magnetic Fields in Unprecedented Ways
Magnetic metamaterials are artificial materials engineered to exhibit magnetic properties not found in naturally occurring substances. These materials are typically composed of periodic arrays of subwavelength structures, such as split-ring resonators (SRRs) or metallic wires, that interact with magnetic fields in unique ways. By carefully designing the geometry and arrangement of these structures, researchers can control the effective permeability, permittivity, and refractive index of the metamaterial, enabling a wide range of functionalities.
One of the most exciting applications of magnetic metamaterials is the cloaking of objects from magnetic fields. By surrounding an object with a metamaterial shell that bends magnetic field lines around it, the object becomes effectively invisible to magnetic sensors. This technology has potential applications in magnetic shielding, magnetic resonance imaging (MRI), and data storage. Metamaterials can also be used to focus and enhance magnetic fields, creating “hotspots” of high magnetic field intensity. This capability can be utilized in various applications, such as magnetic resonance imaging (MRI) and magnetic hyperthermia for cancer therapy.
Furthermore, magnetic metamaterials can be designed to exhibit negative permeability, a property not found in conventional materials. This allows for the manipulation of magnetic fields in novel ways, such as the creation of perfect lenses that can focus magnetic fields with subwavelength resolution. The development of magnetic metamaterials is a rapidly evolving field with immense potential for transforming various areas of science and technology. However, challenges remain in the fabrication of these materials and in scaling up their production for practical applications.
## V. Spintronics: Harnessing Electron Spin for Advanced Electronics
Spintronics, or spin electronics, is a revolutionary field that utilizes the spin of electrons, in addition to their charge, to carry, process, and store information. Unlike conventional electronics, which relies solely on the flow of electric current, spintronic devices exploit the inherent magnetic moment of electrons to achieve faster, more energy-efficient, and non-volatile memory and logic operations. Spintronic devices hold the promise of overcoming the limitations of conventional CMOS technology and ushering in a new era of electronics.
One of the most successful spintronic devices is the spin valve, which consists of two ferromagnetic layers separated by a non-magnetic spacer layer. The resistance of the spin valve depends on the relative orientation of the magnetization of the two ferromagnetic layers. When the magnetizations are parallel, the resistance is low, and when they are antiparallel, the resistance is high. This effect, known as giant magnetoresistance (GMR), is used in hard disk drive read heads to detect weak magnetic fields.
Another important spintronic device is the magnetic tunnel junction (MTJ), which consists of two ferromagnetic layers separated by a thin insulating barrier. Electrons can tunnel through the barrier, and the tunneling current depends on the relative orientation of the magnetization of the two ferromagnetic layers. MTJs are used in magnetic random-access memory (MRAM), which offers non-volatility, high speed, and low power consumption. Ongoing research in spintronics focuses on developing new materials and device architectures to improve the performance of spintronic devices and expand their applications to areas such as sensors, logic circuits, and quantum computing.
## VI. Magnetic Sensors: Enhancing Detection Capabilities
Magnetic sensors are crucial components in a wide range of applications, from automotive systems and industrial automation to medical diagnostics and security systems. These sensors detect and measure magnetic fields, providing valuable information about the environment and the objects within it. Recent advancements in magnetic materials have led to the development of more sensitive, accurate, and compact magnetic sensors.
Hall effect sensors, based on the Hall effect (the generation of a voltage across a conductor carrying current in a magnetic field), are widely used due to their simplicity and low cost. However, their sensitivity can be limited. Magnetoresistive sensors, such as anisotropic magnetoresistance (AMR) sensors and giant magnetoresistance (GMR) sensors, offer significantly higher sensitivity. AMR sensors utilize the change in resistance of a ferromagnetic material with the direction of the applied magnetic field, while GMR sensors exploit the giant magnetoresistance effect in multilayered structures.
Fluxgate sensors are another type of highly sensitive magnetic sensor that utilizes the non-linear magnetic properties of a ferromagnetic core to detect weak magnetic fields. These sensors are often used in magnetometers and navigation systems. Recent research in magnetic sensors focuses on developing new materials and device architectures to further enhance their sensitivity, reduce their size, and improve their energy efficiency. For example, spintronic sensors based on magnetic tunnel junctions (MTJs) offer the potential for ultra-high sensitivity and low power consumption.
## VII. Biomagnetic Materials: Applications at the Intersection of Biology and Magnetism
Biomagnetic materials are materials that exhibit magnetic properties and are designed for applications in the biological and medical fields. These materials can be used for a wide range of applications, including targeted drug delivery, magnetic hyperthermia for cancer therapy, magnetic resonance imaging (MRI) contrast enhancement, and biosensing. The key challenge in developing biomagnetic materials is to ensure biocompatibility, biodegradability, and non-toxicity.
Superparamagnetic iron oxide nanoparticles (SPIONs) are one of the most widely used biomagnetic materials. These nanoparticles exhibit superparamagnetism, meaning that they are magnetic in the presence of an external magnetic field but lose their magnetism when the field is removed. This property prevents the nanoparticles from aggregating and causing blockages in blood vessels. SPIONs can be functionalized with targeting ligands, such as antibodies or peptides, to selectively bind to specific cells or tissues.
In targeted drug delivery, SPIONs can be loaded with therapeutic drugs and guided to the target site using an external magnetic field. In magnetic hyperthermia, SPIONs are injected into a tumor and heated by applying an alternating magnetic field. The elevated temperature kills the cancer cells while sparing healthy tissue. SPIONs can also be used as contrast agents in MRI to enhance the visibility of tumors and other abnormalities. Ongoing research in biomagnetic materials focuses on developing new materials with improved biocompatibility, biodegradability, and magnetic properties, as well as on exploring new applications in diagnostics and therapy.
## VIII. Additive Manufacturing of Magnetic Materials: Shaping the Future of Magnetism
Additive manufacturing, also known as 3D printing, is revolutionizing the way we design and manufacture materials and devices. It allows for the creation of complex geometries and custom designs that are difficult or impossible to achieve with traditional manufacturing techniques. Additive manufacturing is particularly promising for the fabrication of magnetic materials and devices, enabling the creation of components with tailored magnetic properties and intricate shapes.
Various additive manufacturing techniques can be used to process magnetic materials, including powder bed fusion (PBF), binder jetting, and directed energy deposition (DED). Powder bed fusion involves selectively melting or sintering layers of powder material using a laser or electron beam. Binder jetting involves selectively depositing a binder onto layers of powder material to create a solid object. Directed energy deposition involves melting and depositing material using a focused energy beam, such as a laser or electron beam.
Additive manufacturing of magnetic materials offers several advantages, including the ability to create complex geometries, tailor magnetic properties, and reduce material waste. For example, researchers have used additive manufacturing to fabricate complex magnetic cores for transformers with improved performance. They have also used additive manufacturing to create custom-designed magnetic sensors and actuators. Ongoing research in additive manufacturing of magnetic materials focuses on developing new materials and processing techniques to improve the quality and performance of 3D-printed magnetic components. The ability to precisely control the microstructure and composition of magnetic materials through additive manufacturing opens up exciting possibilities for designing and manufacturing novel magnetic devices with unprecedented capabilities.
## Conclusion
The field of magnetic materials is undergoing a period of rapid innovation, driven by the demand for stronger, more efficient, and sustainable magnetic technologies. From novel permanent magnets that push the boundaries of performance to advanced magnetocaloric materials for green cooling and spintronic devices for faster computing, these breakthroughs hold the potential to transform various aspects of our lives. Furthermore, the development of magnetic metamaterials, highly sensitive magnetic sensors, and biomagnetic materials are opening up new avenues in fields ranging from cloaking technology and medical diagnostics to targeted drug delivery. Finally, the advent of additive manufacturing techniques is revolutionizing the way these materials are processed and shaped, enabling entirely new design possibilities. As research continues to advance, we can expect even more exciting developments in the field of magnetic materials, shaping the future of technology and society.
## FAQ
### ### What are the main challenges in developing rare-earth-free permanent magnets?
The main challenges include achieving comparable magnetic performance (energy product and coercivity) to NdFeB magnets, developing cost-effective synthesis routes, and ensuring long-term stability and high-temperature performance.
### ### How does magnetic refrigeration work, and what are its advantages over traditional refrigeration?
Magnetic refrigeration utilizes the magnetocaloric effect to cool materials. By applying and removing a magnetic field, a material undergoes temperature changes that can be used to extract heat from a cold reservoir and release it into a hot reservoir. Advantages include higher energy efficiency, reduced greenhouse gas emissions (no ozone-depleting refrigerants), and lower noise levels.
### ### What are the key properties that define a good soft magnetic material?
Key properties include high permeability (ability to concentrate magnetic flux), low coercivity (resistance to demagnetization), high saturation magnetization (maximum magnetic moment), and low core losses (energy dissipated during magnetization cycles).
### ### What are some potential applications of magnetic metamaterials besides cloaking?
Applications include magnetic field focusing, magnetic lenses for high-resolution imaging, magnetic sensors with enhanced sensitivity, and tunable microwave devices.
### ### How does spintronics differ from conventional electronics?
Spintronics utilizes the spin of electrons, in addition to their charge, to carry, process, and store information, whereas conventional electronics relies solely on the flow of electric current (charge). Spintronics offers potential advantages in terms of speed, energy efficiency, and non-volatility.
### ### What are the main types of magnetic sensors, and how do they work?
Main types include Hall effect sensors (voltage generation due to magnetic field), magnetoresistive sensors (change in resistance due to magnetic field), and fluxgate sensors (exploitation of non-linear magnetic properties).
### ### What are superparamagnetic iron oxide nanoparticles (SPIONs) and why are they used in biomedicine?
SPIONs are iron oxide nanoparticles that exhibit superparamagnetism. They are used in biomedicine due to their biocompatibility, biodegradability, and non-toxicity. They can be used for targeted drug delivery, magnetic hyperthermia, and MRI contrast enhancement.
### ### What are the advantages of using additive manufacturing to create magnetic components?
Advantages include the ability to create complex geometries, tailor magnetic properties, reduce material waste, and accelerate prototyping and manufacturing processes.
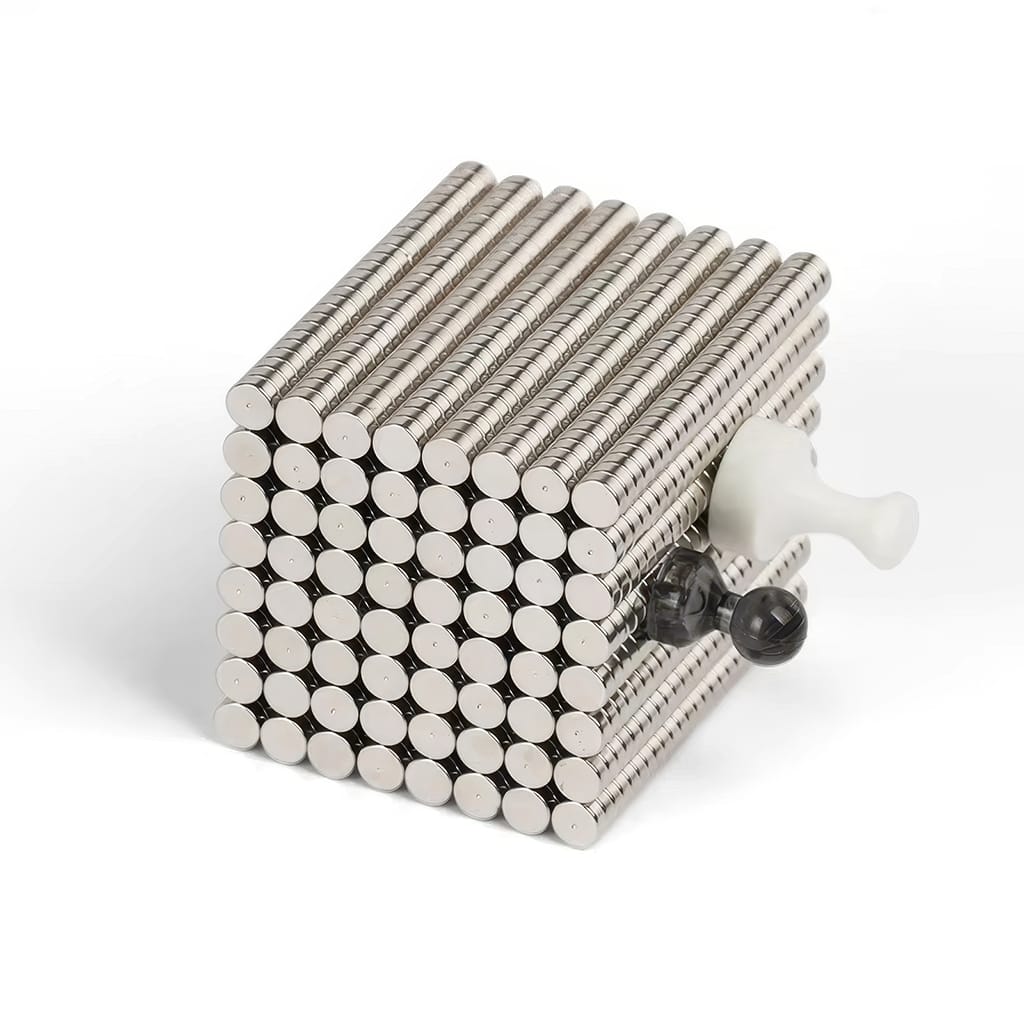