# The Magnetic Frontier: Unveiling the Power of Strong Magnetic Fields
**Summary:** This article delves into the exciting and largely unexplored realm of strong magnetic fields, outlining their current applications, potential breakthroughs, and the profound impact they could have on various scientific and technological domains. From revolutionizing energy production to enabling new medical therapies and uncovering fundamental secrets of the universe, we explore why the “magnetic frontier” is poised to reshape our future. Read on to discover the untapped potential and the scientific hurdles that researchers are racing to overcome.
## Understanding the Landscape of Strong Magnetic Fields
Strong magnetic fields, often defined as fields exceeding a few Tesla (T), an order of magnitude greater than those produced by typical laboratory magnets, represent a unique and powerful tool for scientific exploration. These fields exert significant forces on charged particles and materials, opening up possibilities for manipulating matter and energy in unprecedented ways. While naturally occurring strong magnetic fields exist in celestial objects like neutron stars (with fields reaching trillions of Tesla), generating and controlling such fields in a laboratory or industrial setting presents considerable engineering challenges.
The strength of a magnetic field is crucially tied to its effects. At lower field strengths, we see familiar applications like MRI (Magnetic Resonance Imaging) which relies on manipulating the spin of atomic nuclei. As field strength increases, more exotic phenomena begin to emerge, including the quantum Hall effect, altering material properties, and even potential for manipulating the vacuum itself (theoretically). This transition into the realm of “strong fields” marks a shift from using magnetic fields as a tool for observation to one of active manipulation and transformation.
## The Current Applications of High-Field Magnet Technology
Despite the challenges, strong magnetic fields are already integral to several scientific and technological applications. Nuclear Magnetic Resonance (NMR) spectroscopy, used to determine the structure and dynamics of molecules, relies on high-field magnets to achieve greater spectral resolution and sensitivity. Particle accelerators, such as the Large Hadron Collider (LHC) at CERN, employ powerful superconducting magnets to bend and focus beams of charged particles, enabling scientists to probe the fundamental constituents of matter. Fusion energy research, a promising avenue for clean energy, utilizes strong magnetic fields to confine and compress plasma, creating the extreme conditions necessary for nuclear fusion to occur.
Beyond pure research, high-field magnets are finding applications in industrial processes. For example, magnetic separation techniques are used to purify materials, recycle resources, and remove contaminants from water. Magnetic levitation trains (Maglev) offer high-speed transportation by levitating above the track, eliminating friction and enabling higher speeds. The continued advancements in high-field magnet technology are constantly expanding the range of achievable applications.
## Fusion Energy: Harnessing the Power of Magnetic Confinement
One of the most compelling applications of strong magnetic fields lies in the pursuit of fusion energy. Fusion, the process that powers the sun, offers the potential for a virtually limitless supply of clean energy. The leading approach to achieving controlled fusion on Earth involves confining a superheated plasma (ionized gas) within strong magnetic fields, preventing it from touching the reactor walls and cooling down. This magnetic confinement approach, exemplified by tokamaks and stellarators, requires extremely powerful and precisely shaped magnetic fields.
Tokamaks, the most mature magnetic confinement concept, utilize a doughnut-shaped reactor and a combination of magnetic fields generated by external coils and internal plasma currents to confine the plasma. Stellarators, on the other hand, achieve confinement solely through externally generated magnetic fields, offering potentially more stable and efficient plasma confinement but presenting a more complex engineering challenge. Both approaches rely on superconducting magnets capable of generating fields of several Tesla or even higher to achieve the necessary plasma density and temperature for sustained fusion reactions. The challenges are immense, but the potential reward – a clean and abundant energy source – is driving continued research and development in this field.
## Unveiling Material Properties with Strong Magnetic Fields
Strong magnetic fields offer a unique window into the exotic properties of materials. At high field strengths, the behavior of electrons in materials can be dramatically altered, leading to new and unexpected phenomena. The quantum Hall effect, mentioned earlier, is a prime example. This effect, observed in two-dimensional electron systems at low temperatures and high magnetic fields, reveals the quantization of electrical resistance and provides insights into the fundamental nature of electrons.
Furthermore, strong magnetic fields can induce phase transitions in materials, transforming them into new states with novel properties. Superconductivity, the ability of certain materials to conduct electricity without resistance, can be enhanced by strong magnetic fields in some cases. Similarly, magnetic fields can influence the magnetism of materials, leading to phenomena like spin reorientation and metamagnetism. By studying these effects, scientists can gain a deeper understanding of the electronic structure and fundamental interactions within materials.
## The Quantum Realm: Exploring Fundamental Physics with Strong Magnetic Fields
Beyond material science, strong magnetic fields provide access to the quantum realm, allowing physicists to probe fundamental laws of nature. One area of active research involves the study of quantum electrodynamics (QED) in strong fields. QED, the theory describing the interaction of light and matter, predicts that in extremely strong magnetic fields, the vacuum itself can become polarized, leading to the creation of virtual particle-antiparticle pairs. While these effects are extremely subtle, they can potentially be observable in the ultra-strong magnetic fields of neutron stars or in future high-field laboratory experiments.
Furthermore, strong magnetic fields can be used to search for hypothetical particles, such as axions, which are candidates for dark matter. Axions are predicted to interact weakly with photons in the presence of a magnetic field, leading to the conversion of axions into photons. Experiments using strong magnetic fields and sensitive detectors are actively searching for this conversion process, hoping to shed light on the mystery of dark matter, which accounts for a significant portion of the universe’s mass.
## Medical Applications: Enhancing Imaging and Treatment
While less explored than some other areas, strong magnetic fields hold promise for future medical advancements. Beyond the ubiquitous MRI, which already utilizes strong magnetic fields for detailed tissue imaging, research is focused on exploring new applications. One area of interest is targeted drug delivery, where magnetic nanoparticles carrying medications are guided to specific locations in the body using external magnetic fields. This could potentially allow for more precise and effective treatment of diseases like cancer, while minimizing side effects.
Another potential application lies in transcranial magnetic stimulation (TMS), a non-invasive technique that uses magnetic pulses to stimulate or inhibit brain activity. While TMS is already used to treat depression and other neurological disorders, stronger magnetic fields could allow for deeper and more focused stimulation, potentially leading to new treatments for a wider range of conditions. However, safety considerations are paramount in all medical applications, and further research is needed to carefully assess the potential risks and benefits of using strong magnetic fields in this context.
## The Challenges of Generating and Controlling Strong Magnetic Fields
Generating and controlling strong magnetic fields is a significant engineering challenge. Traditional electromagnets are limited by the saturation of their iron cores, which restricts the maximum achievable field strength. Superconducting magnets, which use materials that conduct electricity without resistance at low temperatures, offer a way to overcome this limitation. Superconducting magnets can generate much stronger magnetic fields than traditional electromagnets, but they require sophisticated cryogenic systems to maintain the necessary low temperatures.
Furthermore, the strong forces generated by high magnetic fields can be immense, putting enormous stress on the magnet structure. Designing magnets that can withstand these forces requires advanced materials and innovative engineering solutions. Another challenge is the control and stability of the magnetic field. Fluctuations in the magnetic field can degrade the performance of experiments and even damage equipment. Sophisticated control systems are needed to maintain a stable and homogeneous magnetic field. Ongoing research is focused on developing new superconducting materials, advanced magnet designs, and improved control systems to overcome these challenges and push the limits of achievable magnetic field strengths.
## The Future of the Magnetic Frontier: A Roadmap for Innovation
The future of the magnetic frontier is bright, with a multitude of exciting research avenues and potential technological breakthroughs. Continued investment in high-field magnet technology is crucial for realizing this potential. This includes the development of new superconducting materials with higher critical temperatures and field strengths, as well as the design of more robust and efficient magnet systems. Furthermore, interdisciplinary collaborations between physicists, engineers, and materials scientists are essential for overcoming the challenges associated with strong magnetic fields.
In addition to technological advancements, fundamental research is needed to fully understand the behavior of matter and energy in strong magnetic fields. This includes theoretical studies of QED in strong fields, experimental investigations of exotic material properties, and the development of new techniques for probing the quantum realm. By pushing the boundaries of knowledge and technology, we can unlock the full potential of the magnetic frontier and pave the way for a new era of scientific discovery and technological innovation.
## Conclusion
Exploring the realm of strong magnetic fields is more than just building bigger magnets; it’s about unlocking a new level of control over matter and energy. From the promise of clean fusion power to the potential for groundbreaking medical therapies and a deeper understanding of the universe, the “magnetic frontier” holds immense potential. While significant technical hurdles remain, the rewards of conquering these challenges are substantial. Continued investment in research and development will undoubtedly shape the future of science and technology for decades to come. The journey into the magnetic unknown is a complex one, but the potential for discovery makes it a voyage well worth undertaking.
## FAQ
### What are Tesla (T) and how do they relate to magnetic field strength?
Tesla (T) is the standard unit of measurement for magnetic field strength in the International System of Units (SI). One Tesla is defined as the magnetic field strength required to exert a force of one Newton on a charge of one Coulomb moving at one meter per second perpendicular to the magnetic field. For context, the Earth’s magnetic field is typically around 0.00005 Tesla (0.5 Gauss), while a typical refrigerator magnet is around 0.01 Tesla. Strong magnetic fields, as discussed in this article, are usually considered to be above a few Tesla, commonly achieved with specialized electromagnets, and especially superconducting magnets.
### Are strong magnetic fields dangerous to humans?
The potential dangers of strong magnetic fields depend on the field strength, duration of exposure, and individual susceptibility. While magnetic resonance imaging (MRI) uses strong magnetic fields (typically 1.5-7 Tesla), it’s considered relatively safe because of strict safety protocols and the controlled nature of the exposure. However, exposure to extremely strong, rapidly changing magnetic fields can induce electric currents in the body, potentially leading to nerve stimulation, muscle contractions, and even cardiac arrest in extreme cases. Metallic implants and devices can also pose a risk in strong magnetic fields. Therefore, careful safety measures, including proper shielding and training, are crucial when working with high-field magnets.
### What are superconducting magnets and why are they important for generating strong magnetic fields?
Superconducting magnets utilize materials that exhibit superconductivity – the ability to conduct electricity with zero resistance – at very low temperatures. This property allows superconducting magnets to carry much larger currents than conventional magnets without significant energy loss due to heat, enabling them to generate much stronger magnetic fields. Keeping them supercooled typically requires liquid helium, which adds to the operational complexity and cost. Recent advances are exploring the use of high-temperature superconductors, which would ideally operate at liquid nitrogen temperatures (or even higher), potentially simplifying cooling requirements. Superconducting magnets are essential for many applications requiring strong magnetic fields, including MRI, particle accelerators, and fusion energy research.
### How are high-field magnets used in particle accelerators like the Large Hadron Collider (LHC)?
In particle accelerators like the LHC, high-field superconducting magnets are used to bend and focus beams of charged particles traveling at near the speed of light. The strong magnetic fields exert a force on the charged particles, causing them to follow a circular path within the accelerator. By increasing the magnetic field strength, the energy of the particles can be increased. Without these high-field magnets, accelerators would need to be orders of magnitude larger to achieve the same particle energies, making them impractical to build. The higher the field strength, the tighter the particles can turn, allowing for circular accelerators of achievable sizes that can reach the very high energies needed to explore fundamental physics.
### What is the quantum Hall effect and why is it important?
The quantum Hall effect is a quantum mechanical phenomenon observed in two-dimensional electron systems subjected to strong magnetic fields and low temperatures. In this state, the electrical resistance is precisely quantized into discrete values, even in the presence of impurities or imperfections. This quantization is remarkably robust and independent of the specific material used. The quantum Hall effect has become a cornerstone of condensed matter physics, providing insights into the behavior of electrons in extreme conditions and serving as a precise standard for electrical resistance. Its applications also extend to metrology and the development of new electronic devices.
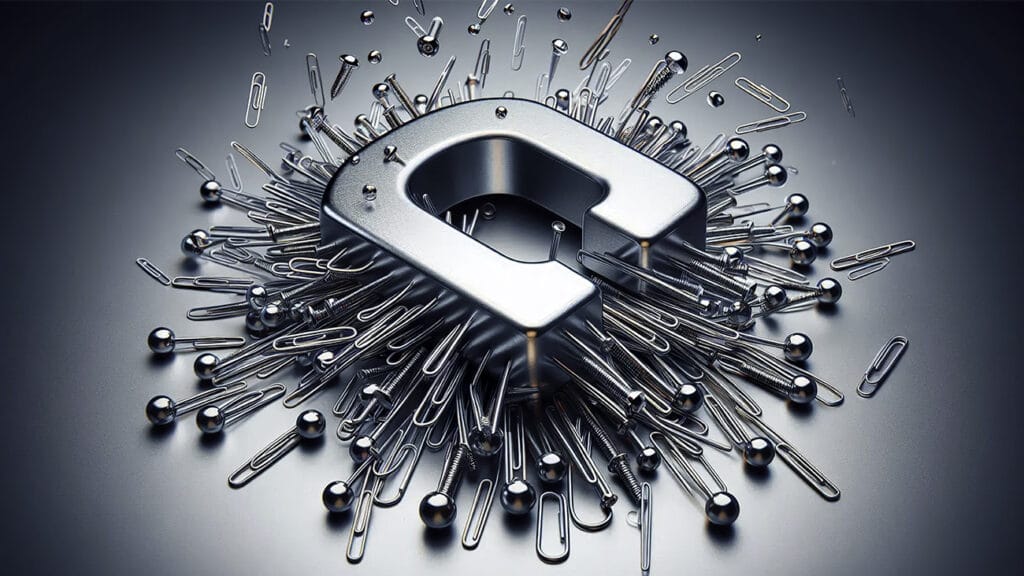